ABOVE: The patch clamp technique ushered in the start of a new era for electrophysiologists, enabling high-resolution recordings of single-channel events. modified from © istock.com, Natali Burma, peterschreiber.media designed by erin lemieux
The intricate machinery of the body, especially how cells communicate and execute crucial tasks in split seconds, has fascinated humans throughout history. In the late 18th century, Italian physician Luigi Galvani jumpstarted the field of electrophysiology when he accidentally caused a dead frog’s legs to twitch with a spark from his metal scalpel.1 This unexpected discovery revealed the vital role of electricity in nerve function.
It was not until the 20th century when researchers appreciated that the body is a biological circuit running on electrical impulses, akin to a game of telephone facilitated via changes in potential differences across the cell membrane.2 To study these electrical signals and wiretap into these neuronal conversations, Kenneth Cole, a biophysicist at the University of Chicago, invented the voltage clamp in the late 1940s.3 He impaled electrodes into rather big cells, such as the giant nerve fibers in squids, which “clamped” the cell membrane at a desired voltage, enabling him to measure currents that passed through the electrode.
In 1952, Alan Hodgkin and Andrew Huxley, physiologists and biophysicists at the University of Cambridge, used Cole’s voltage clamp and measured the first action potential. In the Hodgkin-Huxley model, the duo detailed how neurons transmit electrical signals through ion channels during action potentials; the nerve membrane only allowed potassium to enter the fiber during the resting phase, but allowed sodium ions to flood in when the fiber was excited and passed the signal onto the next neuron.4
Although biophysicist Bernard Katz from University College London proposed the hypothetical idea of single ion channels in 1954, measuring currents at that resolution was not technically possible.5 This inspired researchers to home in on the elusive signals of ion channels. The refinement of voltage clamps gave rise to patch clamp electrophysiology and heralded a whole new world of studying ion channels. This technique deepened the understanding of cellular electrophysiology in a menagerie of cells and their role in diseases.
Electric Dreams: The Birth of Patch Clamp Electrophysiology
The elusiveness of single ion channels intrigued researchers for another quarter of a century. In his youth, Erwin Neher, now a biophysicist at the Max Planck Institute (MPI) for Multidisciplinary Sciences, was fascinated by the idea of electricity in the body, where nerve impulses ran from the brain to the toes. He pursued a career in the biophysics of nerve excitation at the MPI of Psychiatry. Specifically, he studied synaptic mechanisms in motor neurons and ion currents in snail neurons using voltage clamping. There, he met cell physiologist Bert Sakmann, who was also conducting his graduate studies, and they became fast friends. “We believed it might be possible to prove the Hodgkin-Huxley concept by measuring electrical currents at a high enough resolution to see the opening and closing of channels,” remarked Neher.
We believed it might be possible to prove the Hodgkin-Huxley concept by measuring electrical currents at a high enough resolution to see the opening and closing of channels.
—Erwin Neher, Max Planck Institute for Multidisciplinary Sciences
With Neher’s expertise in measuring local currents in muscle cells’ acetylcholine (Ach)-activated channels and Sakmann’s experience in preparing frog neuromuscular junctions, the duo spent the next few years at the MPI for Biophysical Chemistry, Göttingen refining this technique with the goal of measuring single channel events. They calculated the expected electrical current measurements until finally, they generated recordings that appeared to be ion channels, which convinced them that they were on the right track.
Their method was a refined version of the voltage clamp, which circumvented the need to impale the cell with electrodes. Instead, Neher and Sakmann used an extremely fine glass pipette filled with an electrolyte solution and carefully applied slight positive pressure as they approached the cell with the pipette tip. Then, they applied a small amount of suction to make contact between the pipette’s small opening and a patch of the cell’s outer membrane. The process required careful manipulation to create a seal so that only ions could flow from the channel into the one to two micrometer diameter pipette tip. At the time, these “loose” seals had roughly 100 angstroms between the pipette and membrane.
Neher and Sakmann recorded the minute changes in the electrical currents, lasting only 10–100 milliseconds, and demonstrated how these channels functioned by opening (seen as a step change in the current) and closing nerve cell membranes to allow certain ions through one at a time. Together, they measured the first Ach-activated single channel recording across a membrane patch of frog skeletal muscle fibers.
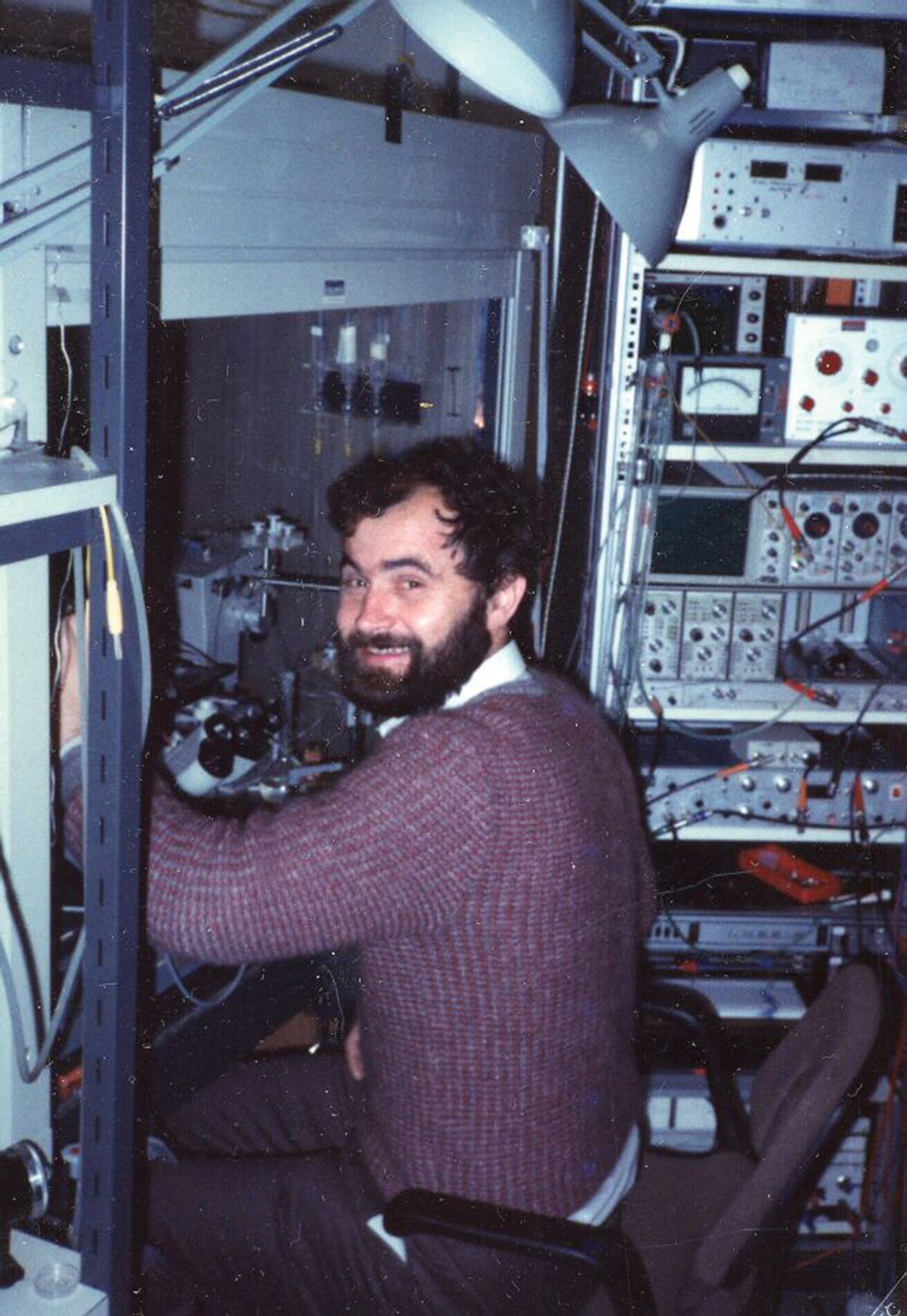
Beginning in 1975, Neher spent a year in the laboratory of Charles “Chuck” Stevens at Yale University during his sabbatical as a visiting scientist. He readied his patch clamp setup and demonstrated more convincing electrical recordings of Ach-activated channels.6,7 “[It] was a real hotbed of exciting work,” recalled Frederick Sigworth, now a biophysicist at Yale University, who joined Stevens’s laboratory as a doctoral student working on noise analysis of ion channel currents. “Around 1976, Erwin Neher performed the very first single channel recording in Chuck’s lab.”
Tangentially, other biophysicists tried to figure out their own early version of the patch clamp method. David Clapham, now a biophysicist at the Howard Hughes Medical Institute, became fascinated with voltage measuring during his time as an MD-PhD student at Emory University. “I really liked the correspondence between biology using electricity. Every cell is like a battery and the ion channels that regulate cells are a bit like transistors, using membrane potential to turn on all kinds of important things,” said Clapham. He joined Louis “Lou” DeFelice’s group at Emory University to study ion channels and membrane noise of the action potentials in cardiac cells.
With conventional voltage clamping, the resulting currents were large, and single channel conductance could only be estimated by the noise analysis that DeFelice had helped to pioneer. When they came across Neher and Sakmann’s paper in 1976, the patch clamp seemed to be a solution. “It got me excited because I wanted to measure the single channels themselves,” said Clapham. Shortly after their breakthrough with patch clamp recordings, Neher returned to the MPI for Biophysical Chemistry, where he shared laboratory space with Sakmann, to further refine the patch clamp. In the early 1980s, Sigworth and Clapham followed Neher across the pond for their postdoctoral research to immerse themselves in the patch clamp technique.
The Power of the Giga Seal: Advancements in Patch Clamp
Neher and Sakmann continued to finetune the patch clamp technique by experimenting with Ach receptors and tinkering with the glass micropipette parameters.8 Their adjoined laboratories quickly became a close-knit group. “It was a very small operation, but it was a really fun time to be working in the lab with everyone. We would walk over to the other end of the institute for lunch and go back for tea. We spent a lot of time interacting,” recalled Sigworth.
Around this time, Sigworth embarked on tackling a very difficult project: measuring sodium channels.9 “Sodium channels are fast, and they have a low conductance, so they would be much harder than acetylcholine receptors,” said Sigworth. He worried about the feasibility of his experiments, but not long after he joined the group, Neher bore promising news.
“It was more or less by chance,” said Neher. While using the loose-seal patch clamping technique, Neher and Sakmann could make repeated measurements, but the loose seal reduced the resistance between the pipette and the membrane. To improve the signal-to-noise recordings, they needed to increase the resistance by tightening the seal.
To create better interactions with membrane lipids, the team altered the charges on the glass and made the pipette tip surface hydrophobic by applying silicone from a Sylgard ball. However, neither of these changes had any major effects. “The only thing that seemed to help was making the pipette tip smaller and applying a bit of suction,” Neher recalled.
It was spectacular. Suddenly, all of the noise [only] came from the amplifier and the electronics themselves.
—Frederick Sigworth, Yale University
While these minute adjustments slightly improved recordings, Neher tried one more tactic: fresh pipettes. Previously, the researchers reused the same pipette. For each experiment, they would first clean the glass pipette, reload the electrolyte solution, and touch the tip to the Sylgard ball. However, when Neher instead used a new pipette to get a seal on a cell, the results perplexed him. “I had a recording going over 10–20 minutes with thousands of openings and closings, but I had no explanation for it,” said Neher. The recordings suddenly showed highly resolved ups and downs. At first, he thought that the pipette was clogged by a particle, but this phenomenon persisted. He realized that using a fresh pipette, applying slight positive pressure before touching the cell, and then using slight suction were key components to attaining a tighter seal.
Neher had stumbled upon a seal that showed improved resistance by a factor of 100, while maintaining the capability to apply voltages to the pipette and have measurable currents. Additionally, he was surprised to find that he obtained this tight seal with hydrophilic surfaces between the fresh pipette and membrane instead of hydrophobic interactions.
“One Monday morning, I came in at 11 o’clock and Erwin said, ‘I think I know how you’re going to see sodium channels’,” said Sigworth. Over the weekend, Neher had mass produced glass pipettes in their microforge. They eagerly tested the pipettes and as they watched the oscilloscope for channel events, the noise recordings diminished as the seal to the glass and the cell membrane became very tight. “It was spectacular,” said Sigworth. “Suddenly, all of the noise [only] came from the amplifier and the electronics themselves.” This was a drastic improvement of resistance from 50 megaohms with a loose seal to a staggering one to five gigaohms with a tightened giga seal.10
“With the loose seal, the distance between the pipette and the membrane was maybe 100 angstroms. With the giga seal, you get within an angstrom or two away and that allowed you to get a much better signal-to-noise ratio,” explained Clapham. He used patch clamp technology to measure membrane area changes and capacitance during vesicle exocytosis, revealing insights into how vesicles, especially neurotransmitters, fuse with and release their contents into the membrane. Later, Clapham went on to patch clamp intracellular organelles such as mitochondria and lysosomes. “It was an exciting time to do those recordings because everything you saw was practically new.”
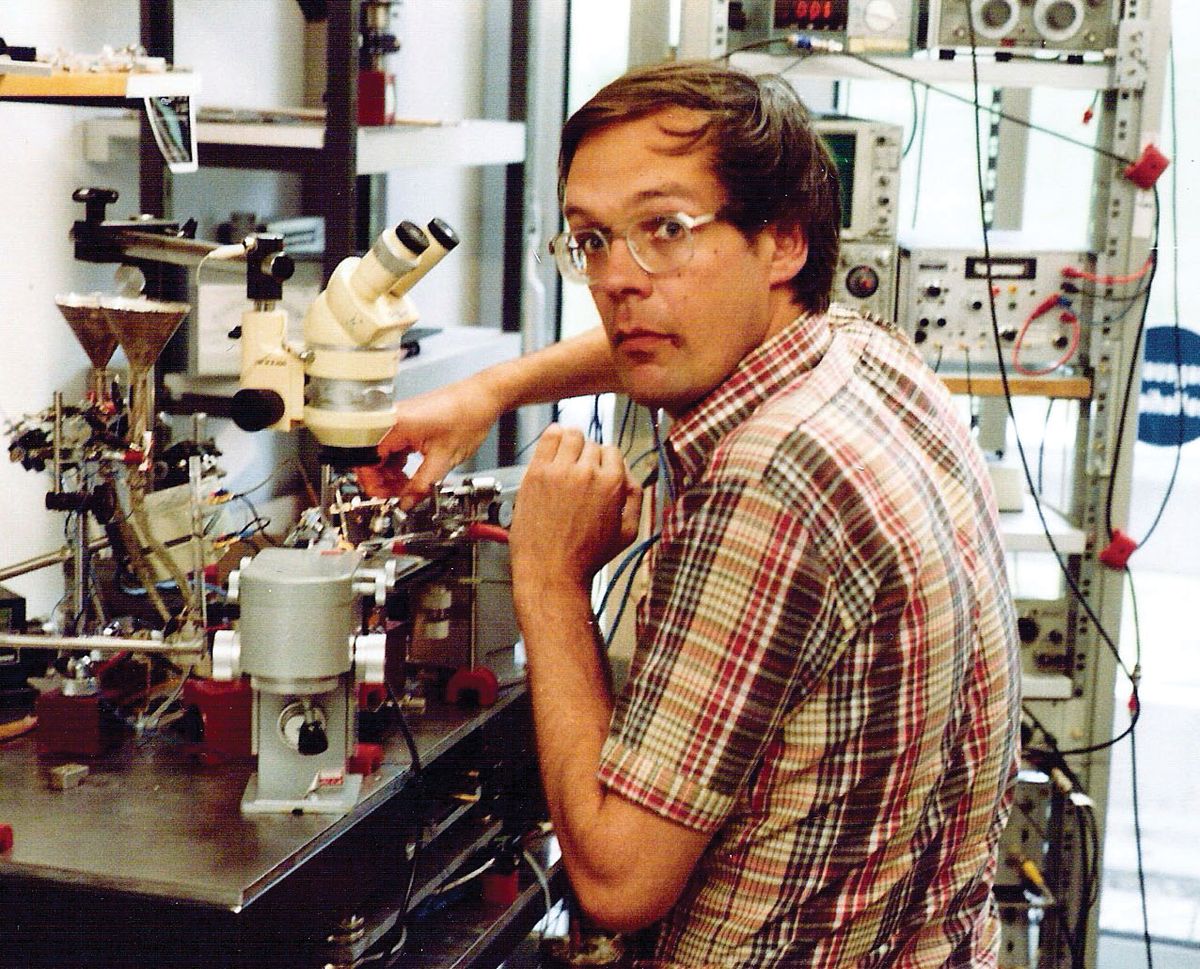
The improvements were two-fold. The giga seal enabled an even higher resolution recording with reduced noise, and Sigworth used his electrical engineering background to build improved amplifiers. The laboratory was abuzz with excitement. “You suddenly had the possibility to record smaller mammalian cells. Electrophysiology switched from using giant cells from strange creatures, such as squids and snails, to more physically representative cells,” remarked Neher.
The next set of discoveries between the Neher and Sakmann labs arrived in a set of three: whole-cell, inside-out, and outside-out patch clamp recordings. Alain Marty, another postdoctoral researcher in Neher’s lab, studied Ach receptors in chromaffin cells. He developed the whole-cell patch clamp technique, where the patch remained attached to the cell and was then broken with a short pulse of suction or a relatively high voltage pulse. This allowed him to measure the voltage or current of the entire cell. “[This] whole-cell recording … in the end, has been the most important version of the patch clamp technique,” said Sigworth. Whole-cell measurement replaced sharp electrodes entirely, which were much more tedious to work with, because the electrodes damaged the membrane when inserted into the cell. “In particular, whole-cell patch clamp revolutionized classical electrophysiology,” remarked Neher.
Subsequently, the groups developed two other configurations that could be performed once they established a seal. For inside-out patches, the pipette was withdrawn while still in a cell-attached state. This motion formed a closed vesicle which remained sealed inside the pipette tip, and the part of the vesicle facing outside the pipette was removed. This configuration exposed the intracellular surface towards the outside of the pipette and was a great method to study channels activated by second messengers such as calcium ions and cyclic adenosine monophosphate. Complementary to this were outside-out patches, in which researchers slowly withdrew the pipette in a way that caused the cell membrane to develop a narrow neck that tied off. This placed the external surface of the membrane on the outside of the patch. This method enabled researchers to manipulate the channels with substances like Ach.
“The giga seal technique and other variations such as inside-out and outside-out patches allowed people interested in ion channels and membrane potential to do a lot more. The field grew tremendously,” said Clapham.
Wired for Success: The Evolution of Patch Clamp Recordings
Since the progression from voltage clamp and patch clamp, this methodological improvement enabled researchers to tune into fast and tiny ion channels at unparalleled resolution. “The electrophysiological approach has its merit for many things,” noted Neher. Over time, researchers found that every cell had ion channels that differed according to what purpose they served, from excitable cells such as neurons, heart cells, and smooth muscle cells to nonexcitable cells.11-14 Researchers have identified more than 300 types of ion channels in the human genome that regulate a wide range of physiological and pathological processes.15 The identification of various ion channels has also advanced researchers’ understanding of ion channel disorders.
The giga seal technique and other variations such as inside-out and outside-out patches allowed people interested in ion channels and membrane potential to do a lot more. The field grew tremendously.
—David Clapham, Howard Hughes Medical Institute
Although patch clamp remains a gold standard workhorse, it comes with its own challenges as the method is labor intensive and suffers from low throughput. To circumvent this, researchers developed automated patch clamp apparatuses for standardized cell lines for drug screening.16 Not only is the technique valuable in biotechnology and pharmaceutical settings, researchers have also adapted patch clamp with complementary technologies.
Some of these combinatory methods include optogenetics, where researchers control the activity of neurons or other cell types with light and track ionic currents and cell membrane potential, and single-cell RNA sequencing (scRNA-seq).17,18 For Cathryn Cadwell, a neuroscientist and physician at the University of California, San Francisco, electrophysiological recordings of neurons are only one facet of these cells.
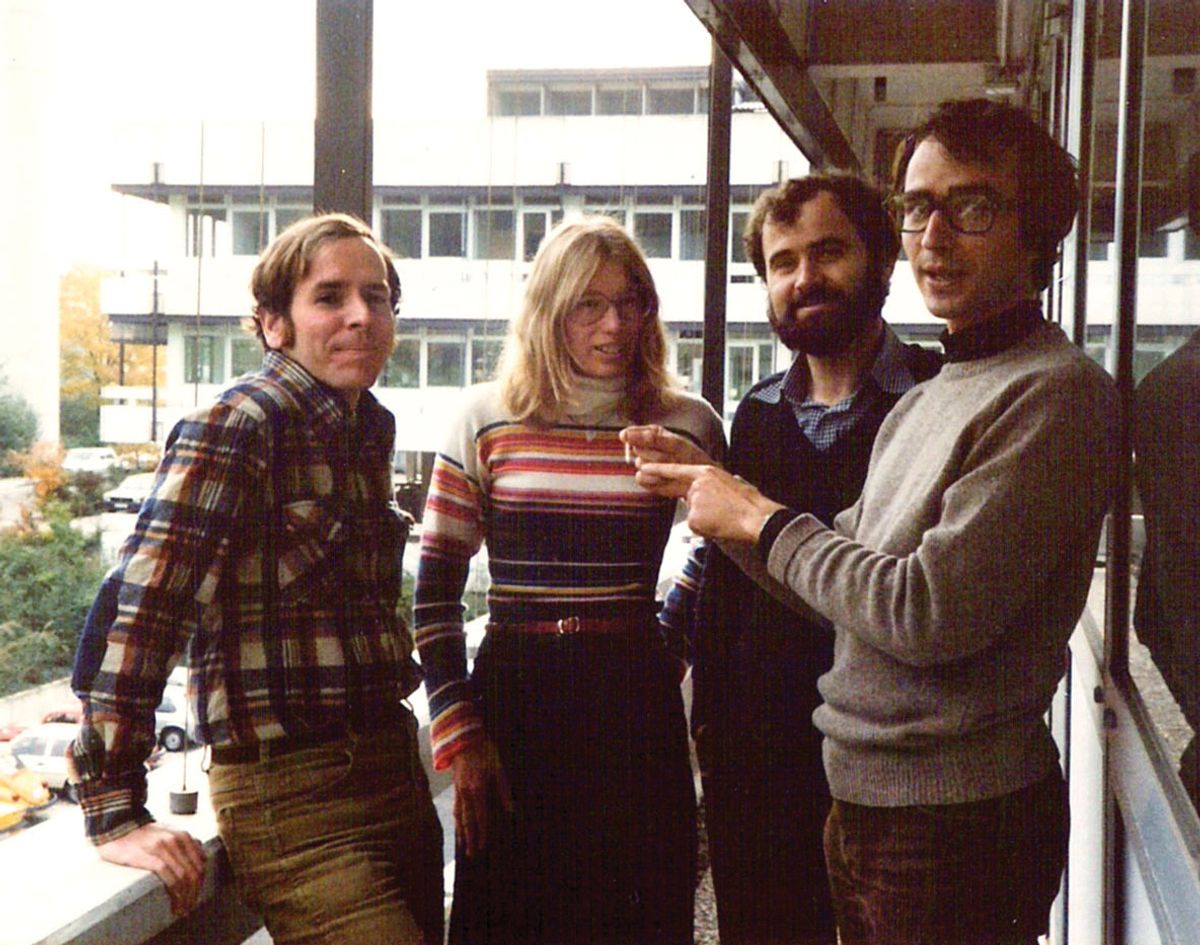
To improve the classification of neuronal cell types and functional characteristics, Cadwell combined whole-cell patch clamp and scRNA-seq to create patch-sequencing (Patch-seq).19 This enabled researchers to measure multiple data modalities in a single cell, such as its electrical properties, morphological phenotype, and transcriptomics.20 “Whole-cell patch clamp is a classic neuroscience technique for studying morphoelectric properties of neurons, and Patch-seq helped integrate these classical phenotypes into modern molecular cell type taxonomies,” said Cadwell. The Patch-seq method helped shed light on aspects of cellular diversity in the nervous system that could not be appreciated using one modality at a time. It has also played an integral role in the BRAIN initiative to characterize cell types across species.
Nearly 50 years since its development, patch clamp remains a tried-and-true method for shedding light on ion channels. “It opened a whole world to directly studying ion channels in real time, and that led to people combining molecular biology and biochemistry with patch clamp…It’s a reliable [technique] that continues to make it possible to discover many more things,” said Clapham.
- Piccolino M. Luigi Galvani and animal electricity: two centuries after the foundation of electrophysiology. Trends Neurosci. 1997;20(10):443-448.
- Hodgkin AL. Evidence for electrical transmission in nerve: Part I. J Physiol. 1937;90(2):183-210.
- Cole KS. Dynamic electrical characteristics of the squid axon membrane. Arch Sci Physiol. 1949;3:253-258.
- Hodgkin A, Huxley A. A quantitative description of membrane current and its application to conduction and excitation in nerve. J Physiol. 1952;117(4):500-544.
- del Castillo J, Katz B. Quantal components of the end-plate potential. J Physiol. 1954;124(3):560-573.
- Neher E, Sakmann B. Single-channel currents recorded from membrane of denervated frog muscle fibres. Nature. 1976;260:799–802.
- Neher E, Sakmann B. Noise analysis of drug induced voltage clamp currents in denervated frog muscle fibres. J Physiol. 1976;258(3):705-729.
- Neher E, et al. The extracellular patch clamp: a method for resolving currents through individual open channels in biological membranes. Pflugers Arch. 1978;375(2):219-228.
- Sigworth F, Neher E. Single Na+ channel currents observed in cultured rat muscle cells. Nature. 1980;287(5781):447–449.
- Hamill OP, et al. Improved patch-clamp techniques for high-resolution current recording from cells and cell-free membrane patches. Pflugers Arch. 1981;391(2):85-100.
- Lai H, Jan L. The distribution and targeting of neuronal voltage-gated ion channels. Nat Rev Neurosci. 2006;7:548–562.
- Bartos DC, et al. Ion channels in the heart. Compr Physiol. 2015;5(3):1423-1464.
- Pereira da Silva EA, et al. Ion channel molecular complexes in vascular smooth muscle. Front Physiol. 2022;13:999369.
- Hamill, OP. Potassium and chloride channels in red blood cells. In: Sakmann B, Neher E, eds. Single-Channel Recording. Springer;2015:451-471.
- Venter JC, et al. The sequence of the human genome. Science. 2001;291(5507):1304-1351.
- Obergrussberger A, et al. Automated patch clamp in drug discovery: major breakthroughs and innovation in the last decade. Expert Opin Drug Discov. 2021;16(1):1–5.
- Linders LE, et al. Studying synaptic connectivity and strength with optogenetics and patch-clamp electrophysiology. Int J Mol Sci. 2022;23(19):11612.
- Tang F, et al.mRNA-Seq whole-transcriptome analysis of a single cell. Nat Methods. 2009;6:377–382.
- Cadwell CR, et al. Electrophysiological, transcriptomic and morphologic profiling of single neurons using Patch-seq. Nat Biotechnol. 2016;34(2):199-203.
- Cadwell CR, et al. Multimodal profiling of single-cell morphology, electrophysiology, and gene expression using Patch-seq. Nat Protoc. 2017;12(12):2531-2553.