FROM TOP (LEFT TO RIGHT): © Science Source/CDC/Phanie; © Science Source/Gary D. Gaugler; © Science Source/Eye of Science; Mary Nora Dickson; © Science Source/David Scharf
Picture a hospital room: white walls, stainless steel IV poles and bedrails, scratchy bedsheets. For more than 100 years, this has been the standard hospital environment, and for most of that time, isolating patients in hygienic rooms, instead of en masse in group clinics or sanatoria, has helped curb the spread of infections that once killed nearly half of soldiers on the battlefield and more than a third of newborn infants. But with the recent rise in antibiotic-resistant pathogens, this standard is no longer sustainable. In 2011, the most recent year data are available from the US Centers for Disease Control and Prevention (CDC), some 720,000 patients acquired an infection while being treated in a health care facility; more than 75,000 of those...
“Imagine one full jumbo jet crashed each day, killing everyone on board,” says Michael Schmidt, vice chairman of microbiology and immunology at the Medical University of South Carolina (MUSC). “This is precisely the number of people that die each day in the U.S. from a hospital-associated infection.”
We can turn almost any material into one that reduces bacterial adhesion and growth, all by implementing nanoscale features.—Thomas Webster,
Northeastern University
In the face of such hospital-acquired, or nosocomial, infections, and the impossibility of developing effective antibiotics quickly enough, researchers are looking to update that white-walled hospital room. The stainless steel IV poles and bedrails could, for example, be coated in nanoparticles of metallic copper, which has antimicrobial properties. Long, thin filaments of nontoxic, bactericidal zinc could provide a protective metallic coating to those scratchy bedsheets, as well as the curtains and paper towels. And the nurse call button and other surfaces could be etched with nanopillars that kill bacteria on contact.
Such nanoscale technologies can decrease the ability of bacteria to adhere to and grow on surfaces by increasing the permeability of bacterial membranes, disrupting protein function, and interfering with cell-cell communication. Moreover, by preventing bacteria from attaching to and colonizing surfaces, these technologies also make it impossible for the microbes to reach a critical population size (the threshold number varies by orders of magnitude depending on the strain) that triggers the formation of a biofilm, which inhibits the entry of antibiotics and disinfectants.
Preliminary in vitro studies have demonstrated that a diverse array of nanotechnologies are effective against some of today’s most dangerous pathogens, such as methicillin-resistant Staphylococcus aureus (MRSA), carbapenem-resistant Enterobacteriaceae (CRE), and Klebsiella pneumoniae. The widespread implementation of these approaches could serve as a stopgap until new therapies are available, and provide additional protection against infection to keep hospitals safe. In addition, nanopatterns designed to be harmless to host cells could be applied to synthetic implants to ward off bacterial growth. As researchers continue to refine and test these approaches against a range of pathogens, the full utility of microbe-resistant materials will become clear.
Click the circles for more information.
See full infopgrahic: WEB | PDF © MATTHEW BROWNSTEIN
Bacteria-resistant surfaces
A few years ago, Schmidt and his colleagues at MUSC tested the idea that an environment coated in copper could stem the spread of infection in a hospital setting. The goal was to harness the antimicrobial power of metallic copper ions, which interact with bacterial surface proteins, damage cell membranes, and are passively or actively uptaken into the cytoplasm. Once inside the bacteria, copper ions form free radicals that damage intracellular proteins and lipids. (See illustration on opposite page.) Copper can even sometimes repel microbes from ever colonizing a surface in the first place. Bacterial membranes and cell walls are studded with proteins that initiate adhesion to surfaces; positively charged copper ions interact with these negatively charged bacterial adhesion proteins to distort protein shape and function, and can outcompete other metals such as zinc that are essential for protein function.
Schmidt and his colleagues refitted several hospital intensive care unit (ICU) rooms using metallic copper alloy surfacing to cover bedrails, IV poles, nurse call buttons, and visitors’ chairs, then randomly assigned patients to either the copper-laden rooms or rooms disinfected using standard protocols. After one year of observation across three separate hospitals, the concentration of bacteria on the surface of the copper-covered objects was a fifth of that on objects in standard ICU rooms, and the rates of nosocomial infection among patients assigned to “copper rooms” were almost 60 percent lower than those in the control rooms.1
However, the MUSC team’s method required that the copper coatings be constructed as full pieces of hardware, which can be prohibitively expensive and time-consuming to install in most modern health care settings. As an alternative, researchers may simply be able to apply metallic nanoparticles to an existing surface to achieve similar antibacterial effects. In 2013, Northeastern University chemical engineer Thomas Webster, president of the US Society for Biomaterials, teamed up with one of his former graduate students to create a selenium nanoparticle spray that can be applied to any surface to cut down on microbial numbers.2 The spray dries within minutes, leaving a layer of antimicrobial nanoparticles behind, and was shown to be nonhazardous in small-animal toxicity studies. Testing the spray on common hospital items, including chairs, bedsheets, and even paper towels, the researchers found that it decreased the overall microbial burden.3 Crucially, the nanoparticles were stable and active until the surfaces were used or washed. “We have seen we can turn almost any material into one that reduces bacterial adhesion and growth, all by implementing nanoscale features,” says Webster.
Another option for preventing microbial growth is to design antibacterial nanoscale features that can be etched into a variety of synthetic materials. Nanoscale pits or troughs can trap bacteria and prevent cell-cell communication, for example. Comparing micron- to nanometer-size troughs, Joanna Verran’s group at Manchester Metropolitan University in the U.K. showed that 200-nm troughs decreased adhesion of three bacterial strains and one yeast strain. As the feature sizes increased (from 500 nm to 2 µm), microbes that preferentially produced biofilms adhered to surfaces more readily: MRSA started adhering at 500 nm, Pseudomonas aeruginosa at 1–3 µm, and Candida albicans at 2 µm.4
SPIKY WINGS: The wings of some cicada species are covered in nanoscale pillars that provide resistance to bacterial infection. Recreating such nanopatterns on synthetic materials could inform the design of antibacterial products. In the 3-D model shown at left (b-e), the outer layer of a rod-shape bacterium (red) begins to rupture against the nanopillars (gray), causing the cell to collapse.© 2013 BIOPHYSICAL SOCIETY. PUBLISHED BY ELSEVIER INC.; © SHUTTERSTOCK.COM/TROPICALPHOTOBANK2Alternatively, nanospikes can kill bacteria by penetrating their cell membranes, controlling microbial growth. In 2013, for example, Albert Yee’s team at the University of California, Irvine, showed that nanopillars that mimic the texture of a cicada wing aid in killing gram-negative bacteria such as Escherichia coli and Klebsiella.5 And in research presented at this year’s American Chemical Society conference in San Diego, the team demonstrated that nanopillars of a slightly different shape modeled after the topography of a dragonfly’s wing are able to kill gram-positive bacteria such as MRSA.6
As an added bonus, such nanopatterned surfaces can often be equipped with functional components, such as biopolymers and antibiotic side chains, that can further decrease the microbial load on a given surface. In a proof-of-concept study, Virginia Davis’s lab at Auburn University in Alabama designed a sheet of antimicrobial single-walled carbon nanotubes (SWNTs). Normally used in electronics development, SWNTs are extremely stable at high temperatures, pressures, and shear stresses, and have a carbon backbone that allowed the team to attach the antibacterial protein lysozyme. In as little as 30 seconds, the material increased bacterial killing by 50 percent compared with nonlysozyme controls.7 Davis and her colleagues formulated sheets of SWNTs as thin as 1.6 nm, allowing the nanomaterial to interact more effectively with bacteria, which have component parts as small as 0.5 nm.
It is quickly becoming clear that using the innate or modified ability of metallic ions and nanopatterned surfaces to kill bacteria or prevent them from forming impenetrable biofilms can be effective and relatively easy. Crucially, pathogens should be unable to evolve resistance to nanosurface strategies of fighting infection: nanoparticle surface energy can always distort protein function on a purely chemical basis, and nanotopographical features will always trap or lyse bacteria. Researchers are now working to develop scalable approaches such as high-throughput etching to tailor nanomaterials to fit large-scale antimicrobial needs.
Nanopatterned devices
Other surfaces that are prone to bacterial growth are those of medical implants, such as hip and knee replacement joints or artificial heart valves. In fact, up to half of all nosocomial infections result from implanted devices, and microbial biofilm growth is a significant cause of implant removal. Moreover, bacteria do not need to be antibiotic resistant to cause an issue, as niches within implants can shelter biofilm-forming microbes from antibiotics and host immune systems.
New nanotechnologies are poised to prevent such problems, however. The use of nanosilver as a coating on embedded medical devices has already demonstrated the ability to inhibit biofilm formation.8 And patterning the plastic or metal surfaces of these implants with nanoscale pillars or pits could similarly decrease the growth of bacterial cells.
Nanopatterned surfaces can also improve host tolerance of the implant, potentially reducing healing time and pain after surgery. Various patterns of blocks less than 10 nm across prevent communication between bacteria, for example, while allowing relatively large, more flexible mammalian cells (~10–120 μm) to adhere. Christopher Bettinger of Carnegie Mellon University and his colleagues showed that long troughs called nanogratings etched into a solid surface promote the elongation of mammalian endothelial cells and eventual blood vessel formation.9 Other research has shown that rough, 50-nm nanotroughs can increase bone formation by osteoblasts while decreasing microbial adhesion and biofilm formation. “Small, long nanofeatures do not allow the somewhat stiff bacteria to attach, yet they allow mammalian cells to function,” explains Webster. These nanopatterned surfaces would be safer in the long run, as mammalian cells take over and grow into vasculature, bone, cartilage, and other tissues.
Alternatively, researchers have used nanopillars like those designed by Yee’s team at UC Irvine to kill any bacteria that land on an implant surface, while leaving mammalian cells unaffected. Bacteria lack cholesterol and other large chemical groups that provide the flexibility to mammalian cells, making bacteria 5 to 20 times stiffer. Thickness of the peptidoglycan cell wall surrounding bacteria can also limit fluidity. As a result, bacteria are punctured by the nanostructures, while mammalian cells are able to “melt” into spaces between nanoscale patterns and grow across the surface.
Nanopatterns designed to be harmless to host cells could be applied to synthetic implants to ward off bacterial growth.
If successfully developed as safe design changes to implanted devices, such nanopatterns may also help prevent microbial spread via surgical instruments. Troublingly, there were 157,000 surgical site infections in the U.S. in 2011 (21.8 percent of all nosocomial infections), and some of them resulted from use of improperly sterilized surgical equipment. In the last year alone, the US Food and Drug Administration (FDA) handed down warning letters to three makers of duodenoscopes for improper sterilization procedures and lack of infection reporting: several people died in numerous hospitals after physicians reused improperly sterilized scopes that passed Pseudomonas aeruginosa and CRE to at-risk patients. In October 2015, the FDA required the companies (Olympus, Pentax, and Fujifilm) to submit new protocols for sterilization procedures.
Within a couple of months, Fujifilm issued revised cleaning instructions for its duodenoscopes and received FDA approval to continue production. And in January 2016, the agency declared that Olympus had provided the necessary modifications to its duodenoscope to prevent leakage of patient fluids into a sealed area inside the device that was harboring hazardous bacteria. At time of writing, Pentax was still working with the FDA to mitigate the potential for cross-contamination due to its devices. None of these companies, however, suggested nanocoatings as a solution. If research continues to show the effectiveness of antimicrobial nanopatterns, it would behoove endoscope manufacturers to consider such technologies. As the steps required for health care personnel to thoroughly clean and sterilize endoscopes between patients are arduous and complicated, nanocoatings in intricate interior chambers of these devices could ensure better microbial control.
Looking to the future
New approaches to discovering antibiotics have received much attention recently, and rightfully so. These techniques are crucial as the numbers of untreatable nosocomial infections continue to rise. But nanotechnological advances to stem such infections are fast becoming a viable strategy to supplement such drug-based approaches. Unfortunately, searches for “nano” and “surface” in clinicaltrials.gov turns up only seven ongoing trials, none of which are related to antimicrobial nanosurfaces. It’s now critical to promote the advancement of nanotechnologies into the clinical setting.
Edward D. Marks is a PhD candidate in the Nanomedicine Research Lab at the University of Delaware. Steven Smith is an infectious disease epidemiologist from the London School of Hygiene and Tropical Medicine.
PROTECTING WATER WITH NANOTECHNOLOGY Beyond preventing the spread of disease in health care settings, antimicrobial nanotechnologies could have much broader applications. Last summer, an outbreak of Legionnaire’s disease in New York City resulted after the infectious agent Legionella pneumophila contaminating cooling towers left more than 100 people hospitalized and 12 dead. The outbreak brought to light how susceptible cooling towers are to biofilm formation and how the towers can broadcast the pathogen as water evaporates into a mist. Similar problems can exist within residential homes, where plumbing systems and hot water heaters can house Legionella colonies, as well as in hospitals, where Legionella colonization of water distribution systems can be aerosolized from whirlpools, nebulizers, oxygen humidifiers, spas, showerheads, and faucets. And unfortunately, once a system becomes contaminated with Legionella, it is virtually impossible to eliminate the pathogen. Efforts to decrease Legionella contamination in both hospitals and cooling towers include thermal disinfection, ozonation, hyperchlorination, UV light sterilization, and the introduction of relatively large (colloidal) particles of copper and silver to the water. While these methods are effective in clearing Legionella organisms, their effects are temporary. Furthermore, the dissemination of colloidal silver particles into water delivery systems can cause birth defects, bluish discoloration of the skin, and diarrhea from gut microflora disruption. Impregnation of fiberglass with a combination of nanometallic particles, such as silver and copper or iron, can be used as a lining within a water delivery infrastructure, especially at faucets, to achieve substantial bactericidal effects. And because the particles are not suspended within the water, they should not have any health effects. Moreover, such an approach would cost less than chemical release systems, as the particles would only need to be installed once, unlike chemicals that must be continuously added to the water. In areas of hospitals serving immunocompromised patients, where any exposure to Legionella could be fatal, such point-of-use filtration using nanosilver was found to eliminate Legionella (BMC Inf Dis, 14:394, 2014). Research on the use of nanosilver in filters continues to probe whether this approach will effectively prohibit the passage of virtually any Legionella organisms at the tap. |
References
- C.D. Salgado et al., “Copper surfaces reduce the rate of healthcare-acquired infections in the intensive care unit,” Infect Control Hosp Epidemiol, 34:479-86, 2013.
- P.A. Tran, T.J. Webster, “Antimicrobial selenium nanoparticle coatings on polymeric medical devices,” Nanotechnology, 24:155101, 2013.
- Results presented at the 2nd International Nanomedicine Conference, Boston, Massachusetts, July 25-27, 2014.
- K.A. Whitehead et al., “Retention of microbial cells in substratum surface features of micrometer and sub-micrometer dimensions,” Colloids Surf B Biointerfaces, 41:129-38, 2005.
- M.N. Dickson et al., “Nanopatterned polymer surfaces with bactericidal properties,” Biointerphases, 10:021010, 2015.
- Results presented at the 251st National Meeting & Exposition of the American Chemical Society, San Diego, California, March 15, 2016.
- D. Nepal et al., “Strong antimicrobial coatings: Single-walled carbon nanotubes armored with biopolymers,” Nano Lett, 8:1896-901, 2008.
- T. Faunce, A. Watal, “Nanosilver and global public health: International regulatory issues,” Nanomedicine, 5:617-32, 2010.
- C. J. Bettinger et al., “Enhancement of in vitro capillary tube formation by substrate nanotopography,” Adv Mater, 20:99-103, 2008.
Interested in reading more?
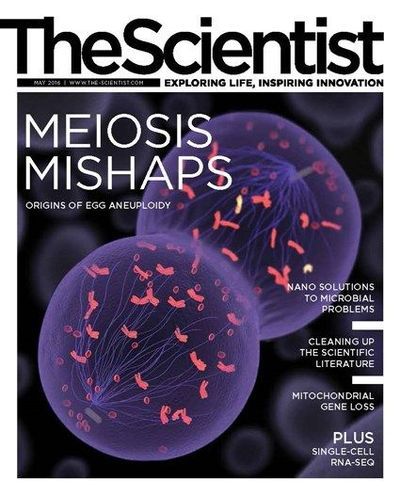